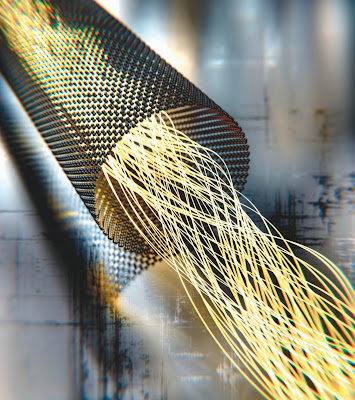 |
Image Source: Link below |
Topics: History, Modern Physics, Quantum Computer, Quantum Mechanics
Soon after Enrico Fermi became a professor of physics at Italy’s University of Rome in 1927, Ettore Majorana joined his research group. Majorana’s colleagues described him as humble because he considered some of his work unexceptional. For example, Majorana correctly predicted in 1932 the existence of the neutron, which he dubbed a neutral proton, based on an atomic-structure experiment by Irène Joliot-Curie and Frédéric Joliot-Curie. Despite Fermi’s urging, Majorana didn’t write a paper. Later that year James Chadwick experimentally confirmed the neutron’s existence and was awarded the 1935 Nobel Prize in Physics for the discovery.
Nevertheless, Fermi thought highly of Majorana, as is captured in the following quote: “There are various categories of scientists, people of a secondary or tertiary standing, who do their best but do not go very far. There are also those of high standing, who come to discoveries of great importance, fundamental for the development of science. But then there are geniuses like Galileo and Newton. Well, Ettore was one of them.” Majorana only wrote nine papers, and the last one, about the now-eponymous fermions, was published in 1937 at Fermi’s insistence. A few months later, Majorana took a night boat to Palermo and was never seen again.1
In that final article, Majorana presented an alternative representation of the relativistic Dirac equation in terms of real wavefunctions. The representation has profound consequences because a real wavefunction describes particles that are their own antiparticles, unlike electrons and positrons. Since particles and antiparticles have opposite charges, fermions in his new representation must have zero charge. Majorana postulated that the neutrino could be one of those exotic fermions.
Although physicists have observed neutrinos for more than 60 years, whether Majorana’s hypothesis is true remains unclear. For example, the discovery of neutrino oscillations, which earned Takaaki Kajita and Arthur McDonald the 2015 Nobel Prize in Physics, demonstrates that neutrinos have mass. But the standard model requires that neutrinos be massless, so various possibilities have been hypothesized to explain the discrepancy. One answer could come from massive neutrinos that do not interact through the weak nuclear force. Such sterile neutrinos could be the particles that Majorana predicted. Whereas conclusive evidence for the existence of Majorana neutrinos remains elusive, researchers are now using Majorana’s idea for other applications, including exotic excitations in superconductors.
Majorana qubits for topological quantum computing, Physics Today
Ramón Aguado is a senior researcher at the Spanish National Research Council (CSIC) in Madrid.
Leo Kouwenhoven is a researcher at the Microsoft Quantum Lab Delft and a professor of applied physics at Delft University of Technology in the Netherlands.